Background
When lasers were first developed, they were referred to as a solution eagerly looking for a problem. Nevertheless, lasers progressed rapidly and became an omnipresent part of modern technology and procedural medicine. Advances in lasers and fiberoptics have made them ideally suited to travel through routes in the human body where no hand or scalpel has gone before. With its widespread use of small-diameter endoscopic instruments, urology has been drastically and positively influenced by this technology, perhaps more so than any other medical subspecialty.
History of the Procedure
Laser is an acronym that stands for light amplification by the stimulated emission of radiation. Albert Einstein proposed the concept of stimulated emission of radiation in 1917. Not until 1960, however, was this theory put to use by T.H. Maimen to produce the first visible-light laser. He used a synthetic ruby crystal with silver-coated ends surrounded by a flash tube to produce light energy. In 1966, Parsons, using a similar ruby laser in a pulsed mode, was the first urologist to experiment with laser light in canine bladders. Mulvany attempted to fragment urinary calculi 2 years later, again using the ruby laser. Subsequently, researchers tested many new substrates or lasing materials, leading to diversity in their clinical application.
Laser physics
Einstein used 2 principles of physics as the basis for his discovery: (1) Light travels in packets of energy known as photons, and (2) most atoms or molecules exist naturally in a ground or low-energy state (E0). However, a small percentage of atoms naturally exists at any given time at a higher, discrete energy level (E1, E2, En). By adding electricity, heat, or light energy to atoms in their ground state, their energy level can be raised. The energy is then released spontaneously in the form of photons or electromagnetic (EM) waves to return to the ground state.
Einstein also discovered that, when a photon of light energy of the same wavelength strikes an excited atom (En), that photon and the photon of light that is released are discharged simultaneously and will therefore be identical in frequency and phase. This is the concept of stimulated emission used in the creation of a laser.
Atoms in their ground state undergo absorption of photons of light energy. For stimulated emission to occur, more atoms must exist in the excited state than in the ground state, a situation known as a population inversion. Energy must be supplied to this population. In a laser, the energy source is usually electric or flashlamp driven. The populations of atoms or molecules that become excited are the lasing medium.
Anatomy of a laser
The lasing medium exists between 2 mirrors for light amplification to occur; one is fully reflective and the other only partially reflective. Once the lasing medium at the core is excited by a pumping mechanism that supplies energy, a population inversion occurs. Some photons are emitted spontaneously from the excited atoms or molecules that cause light to travel in all directions within the laser cavity.
The light that is directed perfectly parallel to the laser cavity is reflected back and forth between the 2 mirrors at their ends. These photons become amplified by collisions with excited atoms in the lasing medium that then release photons in exactly the same direction, phase, and wavelength. The partially reflective mirror at one end has an aperture through which the amplified light exits as a laser beam.
The 3 characteristics mentioned above differentiate laser light from natural light. These include coherence (the photons are all in phase), collimation (they travel parallel with no divergence), and monochromaticity (they all have the same wavelength and, therefore, the same color if within the visible light spectrum).
Different lasing mediums (which can be solid, liquid, or gas) emit photons in different wavelengths of the EM spectrum. This is at least partly responsible for the unique characteristics of a particular laser. Other characteristics that affect laser performance include the power output and the mode of emission (eg, continuous wave, pulsed, or Q-switched).
Continuous wave lasers emit a steady-state, uninterrupted beam. Pulsed lasers have further subdivisions, yet they all allow for more precise control and less lateral heat conduction to tissues than a continuous output laser. A gated pulse laser has a timed interrupted output with a peak power no higher than if the beam were emitted continuously. A true pulse refers to a mode in which the power output is built up between pulses, resulting in a higher peak power than a continuous mode. A superpulse is similar to true pulse; however, the frequency of pulses per second is so fast (about 300-1000/s) that the beam appears to be continuous. Finally, a Q-switched mode refers to a pulsing technique that produces very high peak power outputs (on the order of tens of millions of watts) for very short durations (a few nanoseconds). This allows for minimal lateral heat conduction and a more precise, directed effect.
The physical properties of a laser can be described using 4 key concepts—energy, power, fluence, and irradiance. Energy describes the amount of work accomplished and is measured in joules. Power refers to the rate of energy expenditure and is measured in joules per second, or watts (1 J/s = 1 W). The total energy applied to a given tissue is a function of the power multiplied by the duration of time the tissue is exposed. The fluence, otherwise known as power density, describes the amount of energy delivered per unit area (J/cm2) and is far more important in determining a laser's effect on tissues than total energy delivered.
Irradiance is a term used to describe the intensity of a laser beam, and it is measured in watts per square centimeter. Irradiance is also inversely proportional to the square of the spot size radius. Lenses or optical fibers can manipulate the fluence or power density of a laser. Lenses focus or defocus a beam to change spot size even when the laser is kept at a constant distance from tissue.
Optical fibers used in laser beam delivery often allow for 10-15° of beam divergence upon exit from its tip. This results in defocusing of the beam with increasing distance from the tip of the fiber. Within a 1-inch working distance, laser intensity can change from making incisions (closest, most concentrated spot size) to vaporizing tissue surfaces (slightly defocused) to coagulating proteins (greatest distance). Halving the spot size of a laser beam, while keeping the amount of energy delivered constant, increases the energy density by a factor of 4 (energy density is inversely proportional to the square of the spot size radius; equation = E/[pi][r2]).
Pathophysiology
The biophysics of laser-tissue interactions
Local tissue properties, combined with the wavelength of laser light used, further affect the quality of the laser-tissue interaction. Examples of tissue properties include the density, degree of opacity (eg, quantity of pigments), water content, and blood supply of the tissue. The more dense or opaque a tissue is, the greater the degree of absorption of light energy and the greater the degree of transformation to heat.
Molecules, proteins, and pigments may absorb light only in a specific range of wavelengths. Hemoglobin, for example, absorbs light energy that has a wavelength as high as 600 nm and is translucent to light beyond this range. (The argon laser produces light of 458-515 nm and, therefore, is heavily absorbed by hemoglobin.) Water also absorbs in a specific wavelength range, beginning with a small amount of absorption from 300-2000 nm, at which point the degree of absorption increases rapidly and continues for several thousand nanometers. The CO2 laser produces light in the far infrared spectrum, at 10,600 nm. This is heavily absorbed by water contained in tissue and, therefore, does not penetrate deeply.
Local blood circulation affects the degree of laser energy absorption via 2 mechanisms. First, as mentioned above, the absorptive properties of individual blood components (eg, hemoglobin, water) differ and interact with light in specific wavelength ranges. Second, the circulating blood acts as a heat sink or radiator by transporting absorbed thermal energy away from the site of delivery. This effectively blunts laser power by opposing its local thermal effects.
The wavelength of laser light can be proportional to the depth of penetration into specific tissues. The longer the wavelength, the deeper the expected penetration. Tissue composition and molecular absorption are among several other factors that play into the laser end effect. The neodymium:yttrium-aluminum-garnet (Nd:YAG) laser, for example, produces light in the near infrared region (1060 nm) and penetrates to a depth of approximately 5-10 mm in most tissues (at its wavelength, Nd:YAG is not absorbed by hemoglobin or water in any significant quantity). The CO2 laser with a wavelength of 10,600 nm (longer wavelength, thus should penetrate more deeply) penetrates only to a depth of less than 0.1 mm because its wavelength is very highly absorbed by tissue water. Ultimately, laser energy and tissue characteristics interact in a complex manner that determines the degree of absorption, penetration, reflection, and scattering of laser energy.
Surgeons currently using lasers seek 4 different effects—thermal, mechanical, photochemical, and tissue-welding effects (which is actually mediated through thermal energy). The most common utilization is the thermal effect, whereby light energy is absorbed and transformed into heat. This results in the denaturation of proteins at 42-65°C, the shrinkage of arteries and veins at 70°C, and cellular dehydration at 100°C. Once water has completely evaporated from tissue, the temperature rapidly rises, carbonization then occurs at 250°C, and, finally, vaporization occurs at 300°C.
The mechanical effect results, for example, when a very high power density is directed at a urinary calculus and a column of electrons is freed rapidly at the stone surface. This creates a plasma bubble that swiftly expands and acts like a sonic boom to disrupt the stone along stress lines.
The photochemical effect refers to the selective activation of a specific drug or molecule, which may be administered systemically but is taken up in selected tissues. By activation of the molecule or drug by a specific wavelength of light, the molecule is transformed into a toxic compound(s), often involving oxygen-free radicals that can cause cellular death through destruction of DNA crosslinks. This is a novel approach to destroying superficial skin or mucosal malignant and premalignant lesions. Lasers are ideally suited because of their power and specific wavelength.
Finally, the tissue-welding effect is derived by focusing light of a particular wavelength to induce collagen cross-linking. By adding proteinaceous materials (eg, 50% human albumin, also known as tissue solder) directly to the tissue edges to be welded or a chromophore that absorbs at the laser's wavelength, increased tensile strength and decreased peripheral destruction can be achieved.
Indications
Laser types and clinical applications
This section focuses on the different types of laser energies that have urologic applications and their basic physical properties. The specific urologic applications of each laser type is discussed below in Current Laser Applications.
Ruby laser
The ruby laser was the first visible laser produced using a synthetic ruby crystal surrounded by a flash tube. The laser produces red light at a wavelength of 694 nm. The crystal's lasing properties degrade with high temperatures; therefore, it is best used at low repetition pulse rates, although a short Q-switched mode is now in favor.
The ruby laser is less efficient than more modern lasing materials. The 695-nm emission, however, is highly absorbed by melanin and is currently used in a Q-switched mode to remove pigmented lesions and tattoos, with little scarring. This laser has little use in urology outside of treating cutaneous lesions and removing hair (eg, from perineal skin prior to urethroplasty).
CO
The CO2 laser emits in the invisible far infrared portion of the EM spectrum, at 10,600 nm. It usually is coupled with a visible helium-neon beam for guidance. Its beam is highly absorbed by water; therefore, it vaporizes water-dense tissues to a superficial depth of less than 1 mm. Heat conduction results in thermal coagulation down to a depth of about 0.5 mm, with only small vessels less than 0.5 mm coagulated effectively. The beam is delivered using an articulating arm with mirrors and a hand piece, which can focus or defocus the lens. A waveguide tube can also be used for laparoscopic use.
Neodymium:yttrium-aluminum-garnet laser
Studies in 1961 showed neodymium produced stimulated emissions. The ion (Nd3+) was then used to dope many different crystals. The Y3 Al5 O12 crystal affectionately known as YAG is used commonly today because of its efficiency, optical quality, and high thermal conductivity, which permits high rates of repetition.
The Nd:YAG laser emits a beam at 1064 nm (near infrared) and can be delivered in a continuous, pulsed, or Q-switched mode. The 1064-nm wavelength allows for a relatively deep penetration of as much as 10 mm because this frequency is outside the absorption peaks of both hemoglobin and water. It has good hemostatic (coagulates blood vessels as much as 5 mm in diameter) and cutting properties and is suitable for lithotripsy when Q-switched.
An optical fiber is used for delivery, which may be passed through all types of endoscopes. A sapphire or crystal tip, which decreases backscatter and allows for precise cutting using a direct touch technique, may also be used at the end of an optical fiber.
The frequency-doubled, double-pulse Nd:YAG (FREDDY) laser is a short-pulsed, double-frequency solid-state laser with wavelengths of 532 and 1064 nm. It is a low-power, low-cost laser developed for intracorporeal lithotripsy that has been a subject of recent investigation. Although the FREDDY laser is effective for lithotripsy, it is does not have a soft-tissue application.
Potassium-titanyl phosphate crystal laser
This laser, also known as a potassium-titanyl phosphate (KTP) laser, yields a green visible light beam of 532 nm by passing an Nd:YAG-produced beam (1064 nm) through a KTP crystal that doubles its frequency (thus, halves its wavelength). This light penetrates less than Nd:YAG because of its shorter wavelength and its absorption by hemoglobin. It is used for incisions, resection, and ablation and can be passed through an optical fiber and thus through endoscopic instruments. One disadvantage of KTP laser energy is that tissue carbonization can be observed, rather than a true ablative effect.
Dye lasers
The lasing medium is an organic liquid dye that must be excited optically by another laser or flash lamp. The wavelength emitted depends on the type of dye used, which can be changed or adjusted. The emitted light, therefore, can be tuned to cover a wide spectrum of visible light. In the pulsed mode, this laser is used for lithotripsy and ablation of vascular lesions. The most common dye used is coumarin, which produces a wavelength of 504 nm when excited by a flashlamp. As opposed to a solid-state laser, the dye in the lasing chamber requires replacement, which may be inconvenient and expensive compared with the maintenance of newer laser systems.
Alexandrite laser
This is another tunable laser composed of a chromium-doped mineral known as alexandrite (BeAl2 04). The wavelength range is from 380-830 nm and is strongest at 700-830 nm. This light is absorbed well by melanin; therefore, it can be used for cutaneous lesions. In a 1-ms pulsed mode delivered with an optical fiber, it is used for lithotripsy of pigmented stones. Combined with indocyanine green dye applied to tissues, this laser can also be used for tissue welding.
Semiconductor diode laser
Laser light is produced using light-emitting diodes (LEDs) between reflecting mirrors in a resonator tube. They are smaller, more efficient, and potentially cheaper than most other lasers now in use. Their wavelength can be tuned by adding various elements (eg, aluminum, indium). An 805-nm laser is produced using AlGaAs, and a 1000-nm beam is produced from the active compound InGaAs.
These lasers are currently used for tissue coagulation and thermal treatment of solid organs, including the prostate. In this setting, the laser energy is delivered into tissue with optical fibers and it increases the local temperature. Benign prostate tissue is affected, and, as the denatured protein is reabsorbed over time, bladder outlet obstruction should decrease.
Holmium:yttrium-aluminum-garnet laser
Holmium:YAG (Ho:YAG) is a somewhat recent addition. It consists of the rare earth element holmium doped in a YAG crystal that emits a beam of 2150 nm. This laser energy is delivered most commonly in a pulsatile manner, using a thermomechanical mechanism of action. It superheats water, which heavily absorbs light energy at this wavelength. This creates a vaporization bubble at the tip of a low–water density quartz or silica fiber used for delivery. This vapor bubble expands rapidly and destabilizes the molecules it contacts. This is ideal for lithotripsy of all stone types [1] , as in the image below. The absorption depth in tissue is 1-2 mm, as long as it is used in a water-based medium. This specific light energy provides good hemostasis when used in a pulsed mode of 250 ms duration and at low pulse repetition rate. At higher repetition rates, it may also be used for incisions.
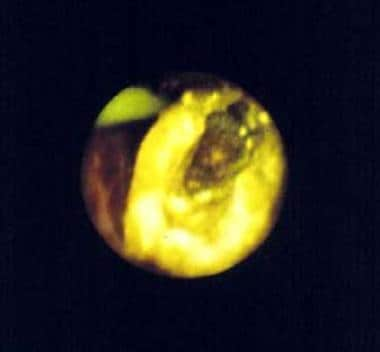
Nitrogen laser
The nitrogen laser incorporates inert nitrogen gas (N2) as the lasing medium. When excited by optical energy, it emits light with a wavelength of 337 nm.
This laser has been studied as a component of a diagnostic test for transitional cell carcinoma (TCC) and other mucosal malignancies using autofluorescence. For this use, the beam is delivered using a quartz optical fiber and the stimulated fluorescence produces light, which is transmitted back through the same fiber to a detection system.
Thulium laser
The thulium:YAG laser has been investigated in an attempt to improve on some of the shortcomings of the Ho:YAG laser. This laser more closely matches the water absorption peak in soft tissue to minimize collateral tissue damage.
One specific application has been in the area of BPH, where thulium vapoenucleation of the prostate (ThuVEP) has been introduced as a size-independent, minimally invasive treatment of BPH using an approach comparable to holmium laser enucleation of the prostate (HoLEP). [2, 3, 4] It offers the advantages of endoscopic, minimally invasive surgical intervention, with the advantages of anatomical blunt dissection of the adenoma like the index finger in open prostatectomy, with a small complication rate. [5, 6] Relative to bipolar resection of the prostate, thulium laser enucleation of the prostate (ThuLEP) is statistically superior in blood loss, catheterization time, irrigation volume, and hospital stay. [7, 8, 9]
The American Urology Association (AUA) recommends both HoLEP and ThuLEP as prostate size–independent options for the surgical management of BPH and as options for patients at a higher risk for bleeding. [10]
Summary of laser types and current clinical applications
See the list below:
-
For soft-tissue incisions (eg, urethral strictures, posterior urethral valves, endopyelotomy, bladder neck contractures), use Ho:YAG, Nd:YAG, or KTP.
-
For resection and ablation (eg, benign prostatic hyperplasia [BPH], TCC, condylomata, penile carcinoma, bladder and skin hemangiomata), use Nd:YAG, Ho:YAG, thulium:YAG, KTP:YAG, semiconductor diode, or CO 2.
-
For lithotripsy (renal pelvis, ureter, and bladder stones), use Ho:YAG, FREDDY, pulsed dye, or alexandrite.
-
For tissue welding (eg, vasovasotomy; urethral reconstruction for hypospadias, strictures, diverticula, or fistulas; pyeloplasty, bladder augmentation, and continent urinary diversion), use diode, KTP, Nd:YAG, or CO 2.
-
For autofluorescence (eg, for diagnosis of bladder malignancies), use a nitrogen laser.
-
For laser hair removal (eg, perineal skin used for local urethral grafts), use ruby, alexandrite, diode, or Nd:YAG.
For urolithiasis, the composition, location, and the size of urinary stones may direct the type of laser and fiber used, the method of approach (eg, retrograde or anterograde), pulsation mode, and power output. With tumors and other lesions, their location, size, and depth will dictate the same parameters.
Relevant Anatomy
Advances in laser and fiberoptic technology have made lasers ideally suited to travel through routes in the human body previously unexplored by hand or scalpel. With the widespread use of small-diameter endoscopic instruments in urology, this field has been drastically and positively influenced by laser technology, perhaps more so than any other medical subspecialty.
-
This is a central stone defect, which is the product of holmium:yttrium-aluminum-garnet (Ho:YAG) laser lithotripsy. This particular stone was composed of cysteine, which will not fragment with the pulsed dye laser. In addition, Ho:YAG produces sulfur dioxide gas when treating cysteine stones, producing a characteristic odor during treatment.